1.11: Periodontium Development
- Page ID
- 41548
\( \newcommand{\vecs}[1]{\overset { \scriptstyle \rightharpoonup} {\mathbf{#1}} } \)
\( \newcommand{\vecd}[1]{\overset{-\!-\!\rightharpoonup}{\vphantom{a}\smash {#1}}} \)
\( \newcommand{\id}{\mathrm{id}}\) \( \newcommand{\Span}{\mathrm{span}}\)
( \newcommand{\kernel}{\mathrm{null}\,}\) \( \newcommand{\range}{\mathrm{range}\,}\)
\( \newcommand{\RealPart}{\mathrm{Re}}\) \( \newcommand{\ImaginaryPart}{\mathrm{Im}}\)
\( \newcommand{\Argument}{\mathrm{Arg}}\) \( \newcommand{\norm}[1]{\| #1 \|}\)
\( \newcommand{\inner}[2]{\langle #1, #2 \rangle}\)
\( \newcommand{\Span}{\mathrm{span}}\)
\( \newcommand{\id}{\mathrm{id}}\)
\( \newcommand{\Span}{\mathrm{span}}\)
\( \newcommand{\kernel}{\mathrm{null}\,}\)
\( \newcommand{\range}{\mathrm{range}\,}\)
\( \newcommand{\RealPart}{\mathrm{Re}}\)
\( \newcommand{\ImaginaryPart}{\mathrm{Im}}\)
\( \newcommand{\Argument}{\mathrm{Arg}}\)
\( \newcommand{\norm}[1]{\| #1 \|}\)
\( \newcommand{\inner}[2]{\langle #1, #2 \rangle}\)
\( \newcommand{\Span}{\mathrm{span}}\) \( \newcommand{\AA}{\unicode[.8,0]{x212B}}\)
\( \newcommand{\vectorA}[1]{\vec{#1}} % arrow\)
\( \newcommand{\vectorAt}[1]{\vec{\text{#1}}} % arrow\)
\( \newcommand{\vectorB}[1]{\overset { \scriptstyle \rightharpoonup} {\mathbf{#1}} } \)
\( \newcommand{\vectorC}[1]{\textbf{#1}} \)
\( \newcommand{\vectorD}[1]{\overrightarrow{#1}} \)
\( \newcommand{\vectorDt}[1]{\overrightarrow{\text{#1}}} \)
\( \newcommand{\vectE}[1]{\overset{-\!-\!\rightharpoonup}{\vphantom{a}\smash{\mathbf {#1}}}} \)
\( \newcommand{\vecs}[1]{\overset { \scriptstyle \rightharpoonup} {\mathbf{#1}} } \)
\( \newcommand{\vecd}[1]{\overset{-\!-\!\rightharpoonup}{\vphantom{a}\smash {#1}}} \)
\(\newcommand{\avec}{\mathbf a}\) \(\newcommand{\bvec}{\mathbf b}\) \(\newcommand{\cvec}{\mathbf c}\) \(\newcommand{\dvec}{\mathbf d}\) \(\newcommand{\dtil}{\widetilde{\mathbf d}}\) \(\newcommand{\evec}{\mathbf e}\) \(\newcommand{\fvec}{\mathbf f}\) \(\newcommand{\nvec}{\mathbf n}\) \(\newcommand{\pvec}{\mathbf p}\) \(\newcommand{\qvec}{\mathbf q}\) \(\newcommand{\svec}{\mathbf s}\) \(\newcommand{\tvec}{\mathbf t}\) \(\newcommand{\uvec}{\mathbf u}\) \(\newcommand{\vvec}{\mathbf v}\) \(\newcommand{\wvec}{\mathbf w}\) \(\newcommand{\xvec}{\mathbf x}\) \(\newcommand{\yvec}{\mathbf y}\) \(\newcommand{\zvec}{\mathbf z}\) \(\newcommand{\rvec}{\mathbf r}\) \(\newcommand{\mvec}{\mathbf m}\) \(\newcommand{\zerovec}{\mathbf 0}\) \(\newcommand{\onevec}{\mathbf 1}\) \(\newcommand{\real}{\mathbb R}\) \(\newcommand{\twovec}[2]{\left[\begin{array}{r}#1 \\ #2 \end{array}\right]}\) \(\newcommand{\ctwovec}[2]{\left[\begin{array}{c}#1 \\ #2 \end{array}\right]}\) \(\newcommand{\threevec}[3]{\left[\begin{array}{r}#1 \\ #2 \\ #3 \end{array}\right]}\) \(\newcommand{\cthreevec}[3]{\left[\begin{array}{c}#1 \\ #2 \\ #3 \end{array}\right]}\) \(\newcommand{\fourvec}[4]{\left[\begin{array}{r}#1 \\ #2 \\ #3 \\ #4 \end{array}\right]}\) \(\newcommand{\cfourvec}[4]{\left[\begin{array}{c}#1 \\ #2 \\ #3 \\ #4 \end{array}\right]}\) \(\newcommand{\fivevec}[5]{\left[\begin{array}{r}#1 \\ #2 \\ #3 \\ #4 \\ #5 \\ \end{array}\right]}\) \(\newcommand{\cfivevec}[5]{\left[\begin{array}{c}#1 \\ #2 \\ #3 \\ #4 \\ #5 \\ \end{array}\right]}\) \(\newcommand{\mattwo}[4]{\left[\begin{array}{rr}#1 \amp #2 \\ #3 \amp #4 \\ \end{array}\right]}\) \(\newcommand{\laspan}[1]{\text{Span}\{#1\}}\) \(\newcommand{\bcal}{\cal B}\) \(\newcommand{\ccal}{\cal C}\) \(\newcommand{\scal}{\cal S}\) \(\newcommand{\wcal}{\cal W}\) \(\newcommand{\ecal}{\cal E}\) \(\newcommand{\coords}[2]{\left\{#1\right\}_{#2}}\) \(\newcommand{\gray}[1]{\color{gray}{#1}}\) \(\newcommand{\lgray}[1]{\color{lightgray}{#1}}\) \(\newcommand{\rank}{\operatorname{rank}}\) \(\newcommand{\row}{\text{Row}}\) \(\newcommand{\col}{\text{Col}}\) \(\renewcommand{\row}{\text{Row}}\) \(\newcommand{\nul}{\text{Nul}}\) \(\newcommand{\var}{\text{Var}}\) \(\newcommand{\corr}{\text{corr}}\) \(\newcommand{\len}[1]{\left|#1\right|}\) \(\newcommand{\bbar}{\overline{\bvec}}\) \(\newcommand{\bhat}{\widehat{\bvec}}\) \(\newcommand{\bperp}{\bvec^\perp}\) \(\newcommand{\xhat}{\widehat{\xvec}}\) \(\newcommand{\vhat}{\widehat{\vvec}}\) \(\newcommand{\uhat}{\widehat{\uvec}}\) \(\newcommand{\what}{\widehat{\wvec}}\) \(\newcommand{\Sighat}{\widehat{\Sigma}}\) \(\newcommand{\lt}{<}\) \(\newcommand{\gt}{>}\) \(\newcommand{\amp}{&}\) \(\definecolor{fillinmathshade}{gray}{0.9}\)- Overview
- Cementum
- Development
- Types of cementum
- Alveolar bone
- Periodontal ligament
- Cells
- Fiber groups
- Gingiva
- Clinical considerations
Figure 11.1: HERS and cementum formation
Overview
The periodontium includes cementum, periodontal ligament, alveolar bone and gingival tissues. The gingiva contain a stratified squamous epithelium which develops from the ectoderm of the pharyngeal arches. Underlying connective tissue layers develop from mesoderm (as does most of the skull). The ECM of these tissues contains collagen and elastin fibers. Cementum, PDL and alveolar bone develop from the neuro-mesenchymal stem cells of the dental sac. These three tissues share extracellular components, making for a strong connection, thanks to their shared lineage. Like mesodermal-derived connective tissues, these tissues have a lot of collagen, but instead of elastin these tissues produce a special fiber type called Oxytalan. Oxytalan fibers can be found in a few other places in the human body, such as the aorta, whose lining is also derived from neural crest cells. Hertwig’s Epithelial Root Sheath plays an important role in the induction of these tissues, even if it is mostly fated to undergo apoptosis.

Cementum development
Cementum forms a thin layer on the roots of teeth, attaching the teeth to the alveolar bone by way of the PDL. The mineral content of cementum is lower than that of dentin, making it appear more radiolucent than dentin, but more radiopaque than the PDL. The surface of cementum will feel grainier than enamel when probed with instruments.

After crown formation is nearly complete, HERS grows apically, separating the dental papilla from the dental sac. The IEE of HERS induces the differentiation of odontoblasts, which begin dentinogenesis. Without stellate reticulum, the IEE cannot be reciprocally induced to form ameloblasts. Instead, the IEE and OEE secrete morphogens onto the surface of dentin, including members of the FGF and Wnt family, but after that, these cells mostly undergo apoptosis. A few epithelial cells will remain and become trapped within the PDL, known as the Epithelial Rests of Mallasez. Some of the epithelial cells of HERS may undergo an epithelial-to-mesenchymal transition and become cementoblasts. With HERS out of the way, pre-dentin made by root odontoblasts now comes into contact with neuro-mesenchymal stem cells of the dental sac. Contact with pre-dentin and with morphogens secreted by HERS induces the neuro-mesenchymal stem cells of the dental sac to become cementoblasts. These cells, like odontoblasts, align up next to one another, behaving more like a tissue derived from ectoderm (an epithelium) than a connective tissue. Cementogensis is the process of forming cementum, its name follows the pattern of amelogenesis and dentinogenesis. Cementoblasts secrete the protein components of cementum. This is frequently referred to as cementoid, which breaks from the pattern we used for pre-enamel and pre-dentin. Layers of cementoid are laid down appositionally, and soon mineralize, at which time it is called cementum. Some cementoblasts become trapped in cementum, after which they are referred to as cementocytes. Other cementoblasts remain near the surface of the cementum. These cells continue to lay down layers of cementoid throughout life, and can become more active during times of injury and repair to cementum. Because of the shared lineage between cementoblasts and odontoblasts, plus the similarity of their ECM, the cemento-dentinal junction (CDJ) is less distinct than the DEJ. In fact, the CDJ was once considered to be imaginary (reviewed here).

One major protein in cementum is collagen, which extends out of cementum into Tomes’ granular layer of root dentin, and in the other direction becomes the collagen fibers of the PDL, which in turn become Sharpey’s fibers in alveolar bone. Rather than thinking about alveolar bone, PDL, cementum and dentin as distinct tissues that are bonded to each other, we should instead think of them as a gradient, thanks to their shared lineage. This makes for a much stronger connection than could be produced by bonding 4 separate tissues, especially ones that are too thin for a good amount of rete peg or dermal papillae-like digitations. Other proteins secreted by cementoblasts include two glycoproteins (bone sialoprotein and osteopontin) which help collagen adhere to calcium hydroxyapatite crystals. Cementoblasts also secrete enzymes that catalyze the formation of crystals, similar to those active in dentinogenesis.

Types of cementum by area of the root.
For most teeth, the apical one third of the root contains cellular cementum, and acellular cementum covers the rest. About 52% of the time, cementum completely covers root dentin, all the way up to the enamel. Roughly 33% of the time there is a gap between the cementum and enamel, with underlying dentin exposed. The rest of the time, roughly 15%, cementum extends over the enamel a short distance. These percentages are not for individual teeth, but for any area of the cervical region on a single tooth. Therefore, a single tooth may have all three patterns. The gap pattern is the most significant because of the increased risk of dentin hypersensitivity. Cementum found overlapping enamel contains no collagen fibers, and does not connect to the PDL.

Periodontal ligament development
The PDL, like other ligaments, is a dense regular connective tissue, composed primarily of collagen fibers in the ECM, plus the special oxytalan fibers. These fibers are made by fibroblasts that differentiate from neuro-mesenchymal stem cells of the dental sac. In figure 260, fibroblast nuclei stain purple. From this image, you should be able to determine which side of the PDL is anchored to bone tissue versus anchored to cementum/dentin based on whether those tissues have cells within lacunae or not. Otherwise, the pink color is mostly collagen found in all 4 of these tissues.

Like other ligaments, the PDL contains osteoblasts (mostly at the border with alveolar bone), and osteoclasts, but unlike other ligaments the PDL also contains cementoblasts and odontoclasts. Furthermore, the stem cells found in the PDL are slightly more differentiated than a typical mesenchymal stem cell, and may be referred to as Periodontal Ligament Stem Cells (PDLSCs). These stem cells can differentiate into fibroblasts, osteoblasts, odontoblasts or cementoblasts given the correct morphogen. This allows the PDL to play a role in the remodeling and repair of bone, cementum and dentin. Whether they are truly distinct from the neuro-mesenchymal stem cells we discussed in the dental sac, dental papilla/dental pulp is unclear, but perhaps relevant to the use of stem cells from extracted teeth to promote the healing of other tissues.

Unlike cementum and dentin, the PDL is vascular. Fibroblasts in the PDL secrete morphogens such as Vascular Endothelial growth Factor (VEGF) to promote angiogenesis. The expression of this gene is controlled by morphogens of the BMP family, which when combined with other morphogens (including FGF), trigger neuro-mesenchymal stem cells to differentiate into fibroblasts. BMPs are also involved in the differentiation of odontoblasts, which is why other morphogen signals are necessary to specify fibroblast differentiation. Fibroblasts in the PDL, which have a neuro-mesenchymal lineage, have a higher capacity to undergo renewal (new ones forming from stem cells) and trigger tissue repair than the fibroblasts derived from mesoderm found in other ligaments. Oddly, the tissues that can best be repaired by cells from the PDL are bone and cementum, not the PDL itself. PDL fibroblasts are distinct from mesodermal fibroblasts in that they express some genes in common with cell types tat share their neuro-mesenchymal lineage. For instance, PDL fibroblasts share features with cementoblasts, expressing RUNX2 and cementoid proteins, as well as neurons, expressing Neuronal Cell Adhesion Molecules (NCAMs) and N-cadherin (a neuronal desmosome protein) (scientific review article).
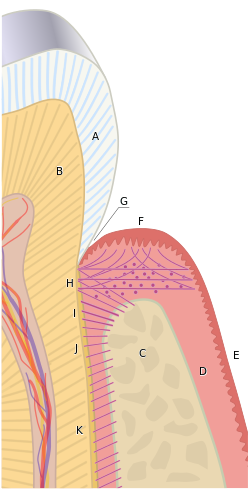
Development of the PDL begins during tooth eruption. PDL Fibroblasts secrete collagen fibers that become embedded in cementum and extend outwards (principal fibers). This process begins at the CEJ and continues apically as the root grows. The other end of collagen fibers is embedded within alveolar bone later, activated by tooth occlusion. This results in greater mobility of tooth roots during eruption. PDL collagen fiber bundles run in different directions, which can be classified as alveolar crest, horizontal, oblique, (peri) apical, interradicular (on multirooted teeth) and trans-septal. You should learn about these different collagen fiber bundles in a different class. Gingival fibers are also collagen fiber bundles, but anchor cementum to the gingiva. These are also categorized based on their location and fiber orientation. Depositing collagen in the correct orientation requires morphogens of the planar cell polarity class. One example is a membrane protein called CD44 which binds to Hyaluronic acid, collagen and fibronectin. These same general types of morphogens are involved in the polarization of odontoblasts following their induction by the IEE. Changes to polarity morphogens are likely necessary for re-orientation of fibroblasts during tooth occlusion and the production of collagen bundles in different directions. When you learn about these fibers, ask your instructor whether the guidance of fibroblast polarity represents the recapitulation of an embryological process.

Remnants of HERS may linger after root formation is finished. These cells, the Epithelial Rests of Mallasez, may be involved in repair of cementum and the PDL (the evidence is not entirely clear at this time). While these cells are epithelial, derived from ectoderm, they may undergo an epithelial-to-mesenchymal transition before differentiating into cementoblasts or fibroblasts, if the correct morphogens are produced. Evidence for this comes primarily from studying transgenic mice, which must be intentionally injured and sacrificed (killed) before repair to tooth tissues can be measured. Obviously, this cannot be repeated in humans.
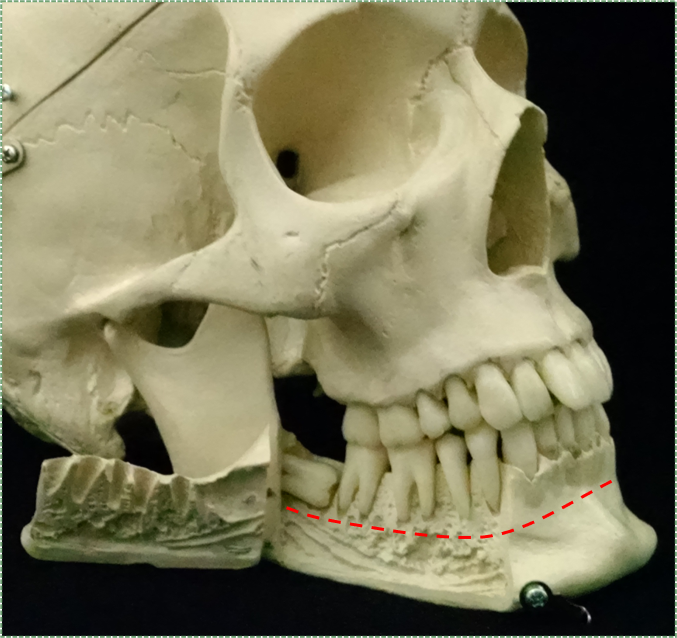
Alveolar bone
The vault of the skull and most of the face develops by intramembranous ossification (including the maxilla). Thus, the sutures contain dense connective tissue (unless they have fully ossified). The base of the skull, on the other hand, develops by endochondral ossification. The development of the mandible is more complicated. First, neuro-mesenchyme forms two bands of cartilage (Meckel’s cartilage). The posterior portion is fated to become the ramus of the mandible plus the malleus and incus bones, while the anterior portion disappears. Before it does, the body of the mandible forms around it by intramembranous ossification. Later, the condylar process, coronoid process and mandibular symphysis develop by endochondral ossification. That is how the skull forms. What is forms from does not match as nicely. Most bones are derived from mesoderm, including the entire appendicular skeleton, plus most of the axial skeleton. The exceptions are the facial bones (including the hyoid) and inferior parts of the cranium, which are derived from neural crest cells. In developmental biology classes, this is not a minor issue: very ancient fish (either long extinct, or modern-day lampreys) have no lower jaw, but they do have seven branchial (pharyngeal) arches. Vertebrates, including you and bony fish, remodel some of the branchial arches into other structures, such as a powerful lower jaw and ears. If you are curios, the lower jaw of a shark (and even the sturgeon) is not a mandible, it is Meckel’s cartilage (so sharks are a closer family member of yours than a lamprey, but more distant relative than a goldfish). This brings us to alveolar bone. The alveolar ridge of both the mandible and maxilla develop from neuro-mesenchyme of the dental sac. Neuro-mesenchymal stem cells in this area are induced to differentiate into osteoblasts by morphogens (including members of the BMP family). This in turn activates transcription factors such as members of the RUNX family and MSX homeobox family, which up-regulate bone-specific proteins. From a development standpoint, it is important to understand that the basal bone of the mandible and maxilla are induced by signals from Meckel’s cartilage, while the induction of alveolar bone formation comes from the developing tooth germ. Alveolar bone contains a number of small Volkman’s canals, through which the PDL connects deeper into bone tissue. This is the same name used for perforating canals that run perpendicular to Haversian canals in compact bone, which contain blood vessels.

Like all bones, the outer portion of alveolar bone is compact bone and sandwiched between that is spongy bone. The compact bone portion is referred to as the lamina dura on a radiograph, as it shows up more radiopaque than the spongy bone deep to it (or the PDL superficial to it). The interdental septum is a triangular-shaped area between two teeth, which should be of uniform height between all teeth. The interradicular septum is the bone tissue between roots
Clinical applications

Hyper-cementosis
Because cementoblasts are retained at the surface of cementum (within the PDL), cementum has the ability to undergo remodeling and repair, similar to dentin but different from enamel. Excessive cementoblast function can result in hyper–cementosis. This more frequently occurs at the root apex due to excessive occlusal forces on a tooth, although it may also be caused by growth factor disturbances such as gigantism/acromegaly or Paget’s disease.

Concrescence
Enough hyper-cementosis can adhere two teeth together by the roots, which is called concrescence. This should illustrate one reason why a radiograph is necessary prior to tooth extraction. This most commonly occurs between the second and third upper molar.

Cemental caries
Gingival recession is worrisome in part because it exposes the roots of teeth to the environment that only enamel is exposed to under healthy conditions. Because of its lower mineral content, cemental caries spread faster than in enamel. Erosion quickly passes to dentin, and if untreated, topulp. Dental pain may not arise until infection of the pulp sets in, at which time treatment is generally limited to tooth extraction rather than prevention and maintenance. Early detection of cemental caries usually involves radiographs or use of a dental mirror ad explorer.
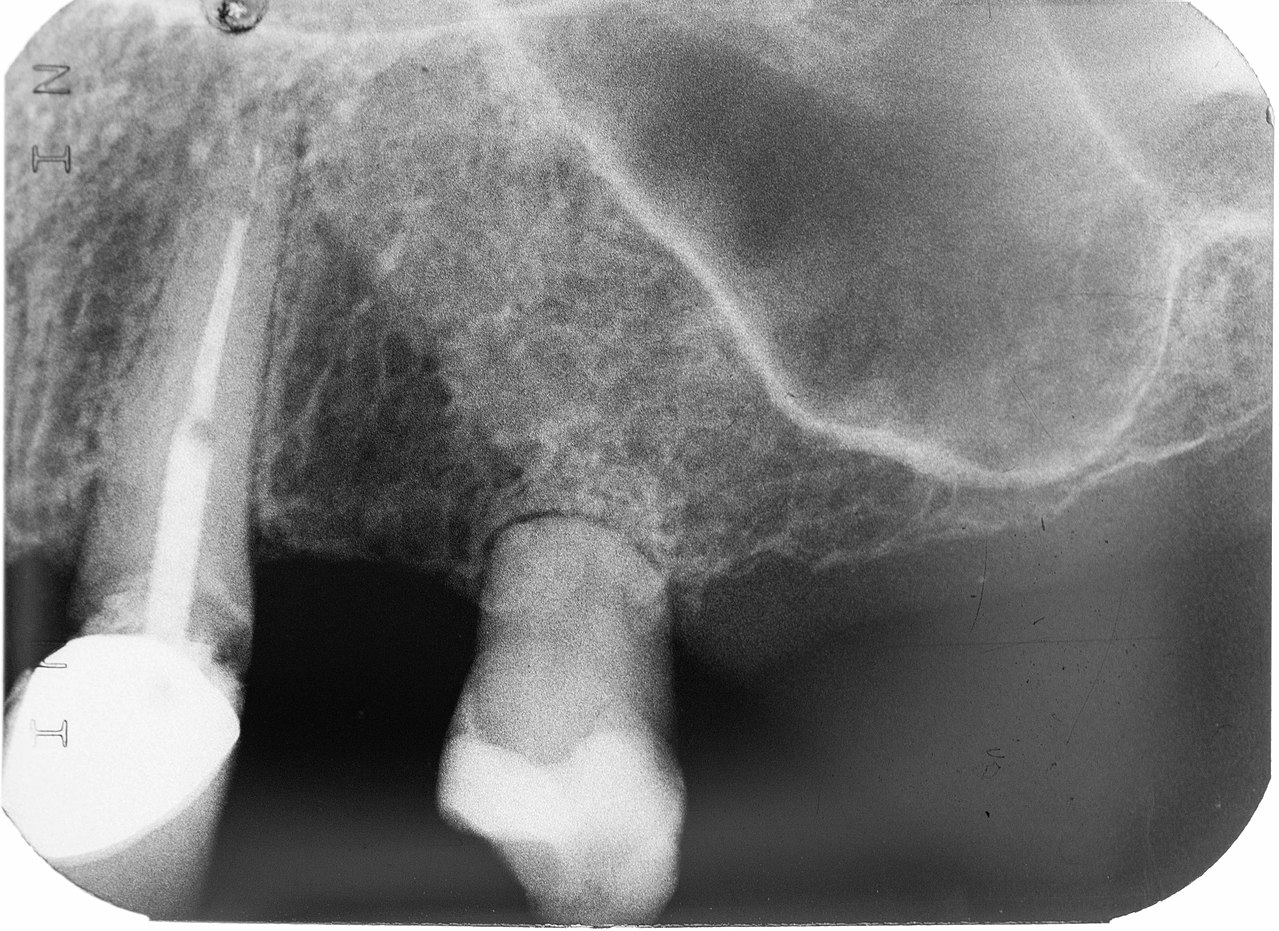
Root resorption
Severe trauma may induce root resorption over time. Both dentin and cementum have the capability to be repaired in response to mild or moderate trauma. This is because living odontoblasts are located in the outer layer of the pulp, and cementoblasts in the PDL. If necessary, mesenchymal stem cells in the pulp or PDL may be induced to differentiate into these cells and lay down more extra-cellular matrix. However, reparative cementum does not contain Sharpey’ fibers and will not contribute to tooth attachment to bone tissue.

Severe trauma, on the other hand, can induce the differentiation of mesenchymal stem cells into cementoclasts or odontoclasts, leading to the loss of cementum and dentin. Loss of these tissues from the roots of teeth is root resorption. Loss of dentin from deep layers is internal resorption. As detin s lost, the space is filled in by vascular pulp tissue, possibly causing the tooth to have a more pinkish coloration. This tooth may be referred to as a pink tooth of Mummery (named for the anatomist who first described the condition, it has nothing to do with a pantomime). If internal resorption is caused by chronic inflammation, removal of inflamed pulp by endodontic therapy may halt the condition. External resorption is the loss of cementum and dentin from the superficial side of the root. External resorption may be transient and resolve on its own, owing to the regenerative capability of cementum thanks to the cementoblasts in the PDL. Chronic inflammation or tooth ankylosis may trigger more severe resorption, where lost tooth tissues are replaced with bone tissue.

Cementicles
Masses of acellular cementum may develop in abnormal locations, especially later in life, and are referred to as cementicles. These may be free (within the PDL but unattached to the tooth), attached (on the surface of the layer of cementum) or embedded (once attached, but now surrounded by the cemental layer of the root). It is thought these develop when cementoblasts encounter debris such as a blood clot (thrombus) lodged in a nearby capillary. This disrupts appositional deposition of cementum and instead creates a nucleus around which a cementicle forms.

Tooth discomfort following endodontic therapy
After endodontic therapy, the pulp—including nerve endings– is replaced with a non-living polymer. Nevertheless, tooth discomfort may still occur. This is because the PDL is still present and living. Besides functioning to attach the tooth roots to bone and gingival tissue, the PDL is involved in proprioception, or the sensation of where the body is located. Nerve endings in the PDL relay information to the somatosensory cortex about where the teeth are located and when they experience occlusal forces. This is very important in reducing or avoiding occlusal forces during mastication and speech. The jaws exert some of the largest amount of force the human body can generate, but also exhibit the greatest amount of control. It is routine for people to bite down through substances of various density and thickness without teeth hitting one another thanks to a large region of somatosensory cortex dedicated to signals from the teeth. Dental implants, however, do not enjoy this sensitivity.

Mesial drift
Mesial drift (or physiological drift) is the tendency of teeth mesial to the first molar the first to migrate in a mesial direction with age. It is a natural phenomenon caused by the remodeling of alveolar bone tissue, which occurs asymmetrically. With age, teeth tend to wear down at their contact points, which would lead to gaps between the teeth. Mesial drift leads teeth to move closer together and prevents gaps from forming despite wear. Too much movement can lead to crowding. The molars, on the other hand, tend to drift distally. Missing teeth can accelerate drift, causing neighboring teeth to bend inwards, changing the bite plane. This, in turn, can lead to abnormal forces in the temporo-mandibular joint, causing pain, discomfort and reduced range of motion.

Loss of alveolar bone
The loss of alveolar bone leads to an uneven level of the inter-radicular septa or inter-dental septa. The consequence of this is a reduced connection between tooth and bone, which can lead to tooth mobility and loss. This is often first noted in the reduced opacity of the alveolar crest region(s). Under healthy conditions, alveolar bone—especially spongy bone—undergoes bone remodeling. Chronic inflammation, such as result of periodontitis, inhibits the rate of osteoblast function without reducing the rate of osteoclast function. This is likely due to the fact osteoclasts are related to white blood cells, their lineage is from the bone marrow—and white blood cells tend to be more active during inflammation, unlike most other cells in the body.
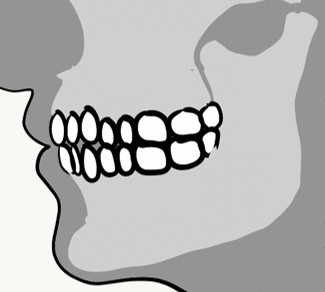
Fenestration and dehiscence
With significant alveolar bone loss, portions of tooth roots may become exposed. A small window of root may become exposed, known as a fenestration (for the Latin word for window). If the exposed root area connects all the way to the CEJ, the region is known as a dehiscence (a general term for a wound that cannot close). This is distinct from gingival recession or periodontal pockets, where junctional epithelium migrates to a region more apical on the root of the tooth, exposing cementum. A fenestration or dehiscence may still be covered by oral mucosa, but the lack of epithelial attachment means oral bacteria may come into contact with cementum and bone tissue, neither of which is designed to resist infection the way partially keratinized avascular epithelia are. This occurs much more frequently on the facial side than the lingual side.

Guided Tissue Regeneration
Surgery can help the body to repair bone loss, including alveolar bone, by filling in the damaged area with a scaffold. The proper scaffolding material allows mesenchymal stem cells to migrate across and differentiate into osteoblasts. Surgeries involving this method are referred to as Guided Bone Regeneration or Guided Tissue Regeneration (GTR). First, a membrane must be placed over the injured area. Old-fashioned membranes, such as cotton gauze bandages, are a barrier between pathogens in the environment and exposed vascular tissues. They also keep other tissues, such as epithelia or scar tissue, from filling up the lost region with the wrong type of tissue. Healing of the injury will occur from the edges of the wound. Newer membranes can be made of molecules that are recognized by mesenchymal stem cells and used as a scaffold over which the cells migrate. These membranes allow for healing to occur across the entire injured area, rather than be limited to the edges. These membranes may be organic (Carbon-containing molecules similar to collagen) or inexpensive inorganic polymers. Furthermore, these membranes may be gelatinous, molded into the injured area, or harder and 3-D printed to fir into the injured area. Bio-active membranes add morphogens that promote the differentiation of mesenchymal stem cells into osteoblasts, rather than fibroblasts (which would produce scar tissue). Similarly, morphogens (such as VEGF) may be included to promote angiogenesis, speeding up the healing process. Antimicrobial agents may also be embedded within the polymer. One consideration when choosing a membrane is whether it is resorbable, or whether it will need to be removed (requiring another surgery). Both organic and synthetic polymers may be resorbable or not. For instance, cotton is organic, but is not resorbed readily by the human body, whereas poly-lactic acid (PLA) membranes (which are also organic, but synthesized in a laboratory) are readily absorbed during the healing process and are replaced by human tissue. Guided tissue regeneration is conceptually very different from replacing damaged tissues with an inert material, such as latex in endodontic therapy, or a filling made of metal, porcelain or plastic—the goal is not to fill in a gap, but to promote the healing of a gap.

Resorption of the alveolar ridge
Following tooth extraction or loss, regions of the alveolar ridge not anchored to PDL and tooth roots undergo resorption. Basal bone should be unaffected. This occurs because all bone tissue undergoes remodeling, and a lack of tension slows down the activity of osteoblasts, but not osteoclasts. The opposite is why exercise maintains healthy bones, which includes the alveolar ridge.

Loss of alveolar bone reduces the vertical dimension of the mandible and/or maxilla. Without teeth to occlude, the mandible and maxilla can move closer together. This causes the mandible to protrudes forward, leading to a “popeye chin”.

Dental implants
Dental implants can prevent loss of alveolar ridge by transmitting force onto bone tissue during mastication. Osteoblasts respond to force by increasing the deposition of calcium and phosphate, increasing bone density and maintaining bone health. If bone loss has already occurred, a bone grafting or guided tissue regeneration procedure may need to be done long enough before the implants are installed to allow for regeneration of lost bone tissue. Similarly, gingival grafting may be involved if gingival tissue has receded significantly.

Dental Implant technology
Each dental implant consists of a core, abutment and crown portion. The core is made of titanium and is implanted into bone tissue. To get the shape and size of the core to properly fit the alveolar ridge of the patient may involve computed tomography (CT scans) to image bone tissue and computer-aided design (CAD) to specify the core’s dimensions. The abutment connects the core to the crown by a screw or dental cement. The crown is usually made of ceramic. Titanium is strong, durable and unlikely to trigger tissue rejection by the patient’s immune system. It is more or less invisible to the cell surface receptors white blood cells use to detect antigens. Unfortunately, it is also invisible to other cells in the body, and as a result does not adhere well to the connective tissues it is embedded in. Knowledge of histology helps with this: coating the core with Hyaluronic Acid improves the connection between the core and the patient’s connective tissue. Another important connection that is lost with tooth extraction is Junctional Epithelium (JE). Because the junctional epithelium of each tooth is derived from the IEE during tooth eruption, new JE does not form on an implant. At best, oral mucosa may adhere to titanium via hemi-desmosomes, creating peri-implant mucosa. Coating a ring of the core with morphogens (such as FGF) can mimic the induction of JE from IEE, improving the connection between oral mucosa and the implant.

Peri-implantitis
Failure to create a complete junction between the oral mucosa and the implant exposes underlying connective tissue to the oral microbiome, frequently leading to peri-implantitis. Because the oral microbiome does not go away, chronic inflammation occurs, leading to loss of tissue around the affected implant, including gingival and bone tissue.
![[crop output image]](https://med.libretexts.org/@api/deki/files/23059/crop-output-image-1.gif?revision=1)
Orthodontia
The same mechanism that leads to both proper bone health and loss of alveolar bone tissue are taken advantage of with orthodontia. Pressure applied to a tooth can cause the PDL on one side to slacken, and on the other side to tense up. The side with lower amount of force will tend to exhibit bone resorption. Higher tension triggers bone deposition on the opposite side. As a result, the location of the alveolar socket shifts in the jawline, without altering the overall dimensions of the socket itself. It is import that the correct amount of force be applied—to much stress can lead to bone resorption all around, increasing the size of the alveolar socket and increasing tooth mobility. Common treatments for osteoporosis include drugs than inhibit the activity of osteoclasts, such as bisphosphonates. Because the entire remodeling unit is required for orthodontia, patients using these medications cannot undergo orthodontic therapy. Another factor to consider with orthodontia is the activity of odontoclasts and cementoclasts. The morphogens that induce odontoclast differentiation and activity (RANKL) during orthodontic movement inhibit the activity of cementoclasts and odontoclasts. For this reason, there is significantly less resorption of cementum and dentin than there is bone tissue during orthodontic movements, even though the change in force across the PDL should be the same for bone and tooth root tissues. It is possible to experience root resorption with orthodontic treatment, especially as the orthodontic force increases, although the causes of root resorption are complex and multi-factorial.

Widening of the PDL space
With occlusal trauma, fibroblasts within the PDL responds with increased activity, leading to a widening of the PDL. For example, in endodontic therapy, a wider PDL space is temporarily generated on the side opposite of the direction of force, but this triggers bone deposition and returns the PDL space to its original width. A wider PDL space can be visualized on a radiograph, and may be accompanied by a thickening of the lamina dura, bone loss, and/or hyper-cementosis. Other conditions besides occlusal trauma may lead to a widening of the PDL space, such as the use of certain medications. The term wider PDL space means that neighboring tissue is lost. It does not necessarily indicate PDL fibroblasts have made more collagen fibers, only that a larger radiolucent gap exists between the root and the lamina dura. This space may contain more ground substance or pus, for instance. This brings us to another example, which may seem contradictory to the first: reduced force can also lead to widening of the PDL space. For example, conditions that negatively affect bone health lead to changes in chewing behavior which in turn reduces occlusal force, followed by bone loss and a widening of the PDL space. Similarly, chronic inflammation of nearby tissues, such as pulpitis, can trigger widening of the PDL space. Whether these conditions stimulate production of collagen by fibroblasts is unlikely because pulpitis and osteoporosis are associated with increased tooth mobility. Common treatments usually aim to reduce the insult, such as using a nightguard if bruxism is suspected. Dental implants help distribute the forces applied to remaining teeth in a partially edentulous mouth, reducing changes to the PDL space and maintaining tooth attachment.

Loss of the PDL apparatus
Loss of attachment between cementum and alveolar bone promotes tooth loss. Two common causes of PDL loss are smoking and bacterial biofilms located within periodontal pockets. With smoking, it is here that nicotine may play a role as a toxin. Nicotine activates nicotinic acetylcholine receptors whose activity modulates blood flow, mitosis, chemotaxis and cell attachment to ECM. These are all necessary for a fibroblast to repair or regenerate the ECM of dense regular connective tissue. Bacterial biofilms contain toxins which can cause similar changes in fibroblast activity—but these toxins generally only diffuse 1-2mm through ECM, which means biofilms not located near the DEJ will be too far away to reach fibroblasts of the PDL (Fig. 11.31).

Regeneration of the PDL following periodontitis
We have discussed regeneration of bone tissue using bioactive scaffolds (guided tissue regeneration), as well as promotion of epithelial attachment to dental implants using ECM protein coatings. The use of similar bioactive membranes to promote regeneration of the PDL has only been studied in animals at this time, not humans. PDLRegeneration is conceptually more complicated—which is perhaps the reason why PDL regenerates poorly, and why we have made less progress on PDL regeneration than bone or oral mucosa. The poor ability of the PDL to regenerate is unusual given it contains stem cells and ample vascularity, two factors that make other connective tissues regenerate well (like pulp, bone and the sub-mucosa of the oral cavity). First, the PDL is polarized, it is not enough to simply boost fibroblast activity. Cells must be oriented correctly to create the proper attachment. Unfortunately, the polarity signals involved in this process are poorly understood at this time.

Secondly, the two connections of the PDL form at different times: collagen fibers are created within cementoid during growth of the roots, but formation of the collagen fibers that connect to alveolar bone do not develop until tooth eruption. Again, the signals involved are poorly understood, and it remains possible that they could be mutually exclusive. We’ve covered the same morphogens doing different things at different times throughout this book. Without knowing more specifics, let us offer a metaphor: the two connections of the PDL could be like car traffic traveling in two directions. For every green light you travel through, cross-traffic must have a red light. With proper timing, you might be able to hit all green lights as you travel down the street, but the traffic light you drove through a minute ago is probably now turning red. It may not be possible to simultaneously get a green light occurring both at the light you are at and the light you were at 5 blocks ago at because urban planners realize that would be a horribly inefficient system.
Don’t worry about the red light 5 blocks behind you, you have the green light to go apply this knowledge to your clinical coursework. If the light turns yellow, yellow means go faster.
Chapter 10 *